By Hadi Zolfaghari and Dominik Obrist
Researchers have used hydrodynamic stability theory for many decades to develop more efficient airplanes, gas turbines, combustion engines, and many other machines. Despite these successes, hydrodynamic stability theory is rarely applied to cardiovascular devices, such as heart valve prostheses. This is a pity, as the study of heart valve prostheses—apart from its societal and medical relevance—leads to many interesting stability problems.
Figure 1. Bileaflet mechanical heart valve. Figure courtesy of
St. Jude Medical.
Work related to hydrodynamic stability and transition to turbulence for heart valves is quite challenging, because researchers must deal with flow pulsatility, decaying turbulence, and fluid-structure interaction; during systole (contraction of the heart), the ejected blood forms a jet within the ascending aorta whose shear layers are unstable and may become turbulent. The rapid deceleration of flow at the end of systole—followed by the valve’s sudden closing—leads to an unorganized flow field with decaying turbulent fluctuations during diastole (relaxation of the heart). Heart valves are mechanically passive structures, and their proper opening and closing is a direct result of the subtle interplay between fluid and structural forces. This fluid-structure interaction may yield instabilities that result in leaflet fluttering, which is akin to a flag fluttering in the wind.
Hydrodynamic instabilities and turbulence past heart valves are also medically relevant phenomena; turbulent blood flow past the aortic valve may cause blood trauma (due to excessive viscous shear stresses) that can lead to blood clots and stroke. Severe leaflet fluttering may generate excessive wear of the valve materials and reduce their durability (especially relevant for valve prostheses made from biological materials). Furthermore, turbulent dissipation in the wake increases pressure loss across the valve, which in turn increases mechanical load on the heart muscle. Finally, turbulent wall shear stresses in the aorta can cause lesions in the vessel wall that may act as the starting points for aortic dissection and aneurysma. Therefore, understanding hydrodynamic instabilities of heart valves—and eventually reducing turbulent flow past the valves—should be a goal of applied fluid mechanics, not only because it is an intriguing flow problem but because there is a medical need for better heart valve prostheses.
Figure 2. Development of systolic flow field (left to right: \(t=\)35, 57, and 79 ms after valve opening). i. Burgers vortex sheets developing at the trailing edge of the leaflets. ii. Vortical flow in the sinus cavities of the aortic root. iii. Stable Falkner-Skan boundary layers at the outer side of the leaflets. iv. Impinging leading edge vortices (ILEVs) with absolute instability at the inner side of the leaflets. v. Interaction between vortical disturbances from the ILEVs and the Burgers vortex sheet, leading to a chaotic breakdown of flow.
Unlike many technical problems that involve Reynolds numbers of several millions or more, blood flows through the aortic heart valve (located between the left ventricle and the aorta) at moderately high Reynolds numbers of only a few thousand. This range of Reynolds numbers falls within the grey zone between laminar and turbulent flow regimes. Therefore, we observe only intermittent phases of turbulent flow in many individuals. In unfavorable configurations (e.g., valvular pathologies, exercise conditions), we may find fully-developed turbulent flows throughout the whole pulse cycle because of increased Reynolds numbers. At the same time, we might find individuals with mostly laminar systolic flow past the heart valve (e.g., small people) because their flow system features lower Reynolds numbers. Doctors utilize this situation at the border between laminar and turbulent flow for the diagnosis of valvular diseases by using a stethoscope to listen for turbulent noise in the ascending aorta.
In a recent study published in Physical Review Fluids, we applied the rich toolbox of hydrodynamic stability theory to a so-called bileaflet mechanical heart valve that features two rigid valve leaflets that freely rotate around a hinge supported by the valve ring (see Figure 1). We chose this valve type over biological prostheses or native human aortic heart valves because it is known to cause unphysiological flow in the aortic root, which is associated with the formation of dangerous blood clots. This valve has a well-defined geometry with only two degrees of freedom of motion, which significantly reduces the problem’s complexity. We further reduced the problem to a two-dimensional configuration with fixed leaflets. High-fidelity simulations of this flow configuration indicate the presence of impinging leading edge vortices (ILEVs) on the inner side of the two leaflets (see Figure 2). Although these ILEVs are known for blunt bodies, previous studies on heart valves have not mentioned them — likely due to insufficient numerical fidelity of the used flow solvers.
Figure 3. 3a. Pocket of absolute instability in the impinging leading edge vortices (ILEVs). 3b. Cusp map for an absolutely unstable flow profile at \(\xi=\)3.2 mm. 3c. Cusp map for an absolutely unstable flow profile at \(\xi=\)2.7 mm.
A local, linear, asymptotic stability analysis of this flow field reveals that the ILEVs feature a pocket of absolute instability (see Figure 3). These theoretical results are consistent with numerical results, which suggest that a wave maker must be associated with the ILEVs (see Figure 2 iv). The simulations also demonstrate that vortical disturbances produced by the ILEV wave maker interact with the Burgers vortex sheets (see Figure 2 i) issuing from the trailing edge of the leaflets. This interaction inspires a rapid breakdown to turbulent flow in the valve’s wake (see Figure 2 v).
Figure 4. 4a. Leaflets with blunt leading edge (original design) and impinging leading edge vortex (ILEV) instability, leading to nonlinear breakdown of the wake. 4b. Leaflets with pointed leading edge and a laminar wake.
These results describe a plausible pathway for the transition from laminar to turbulent flow. And because this pathway starts with the wave maker in the ILEVs, we attempted to reduce turbulence in the wake by eliminating the ILEVs. Numerical simulations with modified geometries show that the ILEV is caused by the blunt leading edge of the leaflets, which are like a symmetric pair of airfoils immersed in the blood flow. We found that pointed leading edge design suppresses the ILEVs, such that the wave maker is eliminated and the laminar-organized structure of the vortex streets in the wake is maintained (see Figure 4).
However, we must remember that these results are based on a simplified two-dimensional model. Therefore, we tested the validity of this simplification by running large, direct numerical simulations of the whole heart valve in an anatomically-correct model of the aortic root (see Figure 5). The Navier-Stokes solver used high-order finite difference schemes on 340 million grid points and a third-order Runge-Kutta scheme. One simulation run required three days on 20 GPUs of a Cray XC40/50 supercomputer. The simulation results helped us identify also other instability mechanisms (e.g., in the sinus cavities, at the leaflet-ring junctions, or flow disturbances from the ventricle), although we found that their contribution to the turbulent breakdown in the wake is small. At the same time, our results confirmed that the ILEV wave maker is the primary instability mechanism, and its elimination leads to significantly reduced levels of turbulence in the wake of the valve.
Figure 5. Direct numerical simulation of bileaflet mechanical heart valve in an anatomically correct aortic root (340 mio grid points; 20 GPUs of Cray XC40/50).
We believe that our study is a sound example of the power of hydrodynamic stability theory applied to biomedical flow problems. It opens the door to further investigations, including global stability analysis, nonmodal stability analysis, and adjoint-based design optimization. This approach may support the development of more efficient, less thrombogenic, and more durable heart valve prostheses.
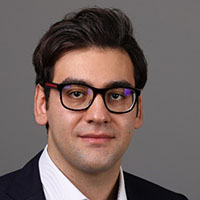 |
Hadi Zolfaghari earned his Ph.D. in biomedical engineering at the University of Bern in March 2020. He is now a postdoctoral fellow at the University of Cambridge. |
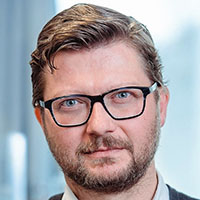 |
Dominik Obrist is a professor of biofluid dynamics and cardiovascular engineering at the University of Bern. |