By Cole Butler
The yellow fever mosquito—also known as Aedes aegypti—is a vector for a variety of dangerous viruses, including those that cause dengue, Chikungunya, Zika, and West Nile. Ae. aegypti mosquitoes are invasive in geographic areas beyond Africa, and more than half of the world’s population is at risk of contracting dengue alone. Though the use of insecticides is the primary method for controlling mosquito populations, insecticide effectiveness has waned in recent years due to rising rates of resistance. However, recent genetic advances have inspired a new technology that has the potential to turn the tables on mosquito-borne disease.
Figure 1. One example of a gene drive (GD) is a so-called “homing” drive. In steps 1 through 3, the GD (in red) “cuts” the chromosome (in green) that is opposite the construct. In step 3, DNA repair mechanisms kick in to repair the broken strand; to do so, they use the unbroken strand of DNA on the homologous chromosome as a template. The GD is then duplicated, as indicated in step 4. Figure courtesy of Cole Butler.
These
gene drives (GDs) can spread a genetic element through a population, even if that GD element is harmful to the target organism. To accomplish this spread, the GD element selfishly biases its own inheritance, which can occur in various ways. One popular technique involves the use of certain genetic complexes that exploit DNA repair mechanisms (see Figure 1). The GD complex “cuts” the homologous chromosome that is opposite to the construct itself, after which DNA repair uses the unbroken chromosome as a template to fix the one that was cut. During the process, the GD complex is copied onto the repaired chromosome and thus duplicated. A mosquito that previously had only one copy of the GD element now has two!
Scientists classify GDs by their intended function: population replacement or population suppression. A population replacement GD can genetically “replace” a wild population. For instance, spreading an anti-pathogen gene throughout a mosquito population would effectively immunize the population against diseases that are harmful to humans. Researchers have already developed such genetic constructs as proof of concept [1]. On the other hand, GDs for population suppression seek to reduce or eradicate pest populations. In principle, this tactic is similar to current population control strategies that involve insecticide use and source control. Population suppression involves attaching a fitness cost to the GD construct or altering a gene that is essential for the organism’s fertility (e.g., individuals with two copies of the GD element become sterile).
Despite this technology’s immense potential, scientists and stakeholders are still uncertain how GDs will behave in the wild. To date, there has never been a field release of a GD-carrying organism. Experimentalists often assess GD performance in carefully controlled laboratory settings, but these trials are far from mimicking the dynamics of a natural setting. Mathematical and computational models are therefore critical for technological development.
Figure 2. A gene drive (GD) that is too deleterious to host fitness relative to host dispersal can “burn out” before it spreads sufficiently far. Here, GD-carrying mosquitoes (in red) are unable to spread the GD to the entire target population. After the GD becomes extinct, the wild-type mosquitoes (in black) recolonize the habitat that is left empty by the construct. Figure courtesy of Cole Butler.
GDs can only travel as far and as fast as the target organisms allow. Because of this limitation, GDs must paradoxically rely on target organisms to survive long enough to spread sufficiently far. A delicate balance thus exists in how deleterious a GD can become before its efficiency declines. Ae. aegypti does not disperse far in its short life [3], so a hypothetical GD that crashes a local mosquito population too quickly might burn out before it spreads an adequate distance (see Figure 2). Local extinction of the GD risks a resurgence in the mosquito population, which could catastrophically affect disease control efforts. This example raises the curious possibility that GD performance may improve if the fitness cost is reduced. In other words, less mosquito killing might be better for long-term control.
The reverse possibility of a GD that spreads too efficiently is also concerning. In such a scenario, the construct could spread beyond its intended target range to non-control regions. A GD is spatially limited if it remains confined to the intended area of control. This condition adds an extra layer of complexity to the problem and begs the following question: How can we design a GD that it is able to spread within a control region but go no further?
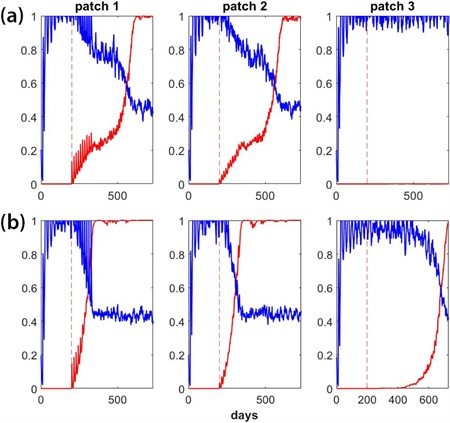
Figure 3. Successful and unsuccessful stochastic gene drive (GD) simulations over the course of two years. Patches 1 and 2 comprise the control region wherein GD spread is desired, while patch 3 represents a non-control region in which GD spread is not desired. Mosquito movement occurs between the patches. Each plot shows the mosquito population relative to equilibrium (in blue) as well as the frequency of the GD construct (in red). The wild-type mosquito population is allowed to reach equilibrium for 200 days prior to GD release. Multiple releases of GD-carrying mosquitoes begin on day 200 (indicated by the dashed red line). 3a. A simple homing GD that remains confined to patches 1 and 2 but does not spread to patch 3. This ideal outcome remains the same even if we increase the simulation time. Note that the mosquito population decreases by half. 3b. The same homing GD but with a reduced fitness cost. In this case, the GD’s fitness cost is too low relative to target organism dispersal. As a result, the GD invades patch 3 (the non-control region). Figure courtesy of Cole Butler.
To answer this query, I have developed a stochastic multi-patch model that incorporates spatial, population, and genetic dynamics. The model uses the
Ae. aegypti mosquito as a target organism and also includes age and sex structure — ecological factors that are difficult to accommodate in laboratory experiments. This model is able to determine whether a GD element can spread locally but remain spatially limited in a mosquito population under realistic conditions. Furthermore, it will allow me to compare the performance of proposed GDs in the literature. Doing so will underline the importance of balancing GD fitness cost with target organism dispersal — a topic that has received comparatively little attention [2].
Preliminary results suggest that it is difficult to balance the GD’s local spread with spatial confinement. Figure 3 compares successful and unsuccessful GD outcomes for a scenario wherein the GD is released in patch 1. Patches 1 and 2 represent adjacent areas in the control region for which GD spread is desired, while patch 3 represents a non-control region in which GD spread is not desired. Mosquitoes disperse between adjacent patches. As Figure 3 illustrates, the relationship between the fitness cost of a GD and target organism dispersal determines overall success or failure. Further study of this relationship will help guide future GD research and highlight the relationship between target organism behavior and GD success.
Cole Butler delivered a contributed presentation on this research at the 2022 SIAM Conference on the Life Sciences (LS22), which took place concurrently with the 2022 SIAM Annual Meeting in Pittsburgh, Pa., last year. He received funding to attend LS22 through a SIAM Student Travel Award. To learn more about Student Travel Awards and submit an application, visit the online page.
SIAM Student Travel Awards are made possible in part by the generous support of our community. To make a gift to the Student Travel Fund, visit the SIAM website.
Acknowledgments: I would like to thank Alun Lloyd and Fred Gould of North Carolina State University for their comments as I was drafting this article.
References
[1] Buchman, A., Gamez, S., Li, M., Antoshechkin, I., Li, H.-H., Wang, H.-W., … Akbari, O.S. (2019). Engineered resistance to Zika virus in transgenic Aedes aegypti expressing a polycistronic cluster of synthetic small RNAs. Proc. Natl. Acad. Sci., 116(9), 3656-3661.
[2] Dhole, S., Lloyd, A.L., & Gould, F. (2020). Gene drive dynamics in natural populations: The importance of density dependence, space, and sex. Annu. Rev. Ecol. Evol. Syst., 51(1), 505-531.
[3] Harrington, L.C., Scott, T.W., Lerdthusnee, K., Coleman, R.C., Costero, A., Clark, G.G., … Edman, J.D. (2005). Dispersal of the dengue vector Aedes aegypti within and between rural communities. Am. J. Trop. Med. Hyg., 72(2), 209-220.
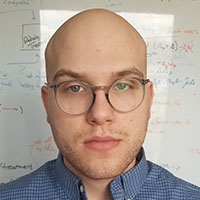 |
Cole Butler spent his childhood getting bit by mosquitoes in Maine. Despite this, he loves the outdoors and studying nature. Butler is a National Science Foundation Graduate Research Fellow and Genetics and Genomics Scholar at North Carolina State University, where he is pursuing his Ph.D. in biomathematics. He earned a B.S. in mathematics from the University of Maine in 2020. Among other things, Butler researches how to best kill mosquitoes. |