Modeling the Spread of Bacteria through Complex Environments
By Sujit Datta
Bacteria critically impact our everyday lives: they cause or prevent disease, foul industrial equipment, spoil foods, degrade contaminants, fix nitrogen and carbon, and decompose organic matter. The ability of individual bacterial cells to spread within their surroundings—either through self-propulsion or by growing, dividing, and pushing their progeny to new locations—underlies many of these processes. Yet despite their ubiquity and importance, the spreading of bacterial populations through real-life complex environments is difficult to mathematically model. Laboratory studies typically focus on bacteria in liquid cultures or at flat interfaces; while these efforts provide powerful insights, they do not adequately replicate the complexity of natural habitats. True habitats are often crowded and porous media, such as tissues or gels in the body and soils or sediments in terrestrial environments. Investigations of this knowledge gap reveal fascinating new questions at the interface of applied mathematics, biology, physics, and engineering.
Animation 1. Movie taken using confocal microscopy of E. coli with fluorescently labeled flagella (magenta) in a three-dimensional porous medium. The cell becomes trapped when it encounters an obstruction; however, the flagella maintain their rotation as a coherent bundle for much longer than the unconfined run duration. The cell continues to reorient itself while trapped, eventually allowing the flagella to unbundle and re-bundle in a different configuration. This outcome enables the cell to escape its trap and resume hopping through the pore space in a different direction. Animation courtesy of [5].
To better understand the movement of bacteria,
our lab at Princeton University developed a “porous petri dish” that enables us to (i) directly visualize bacterial spreading at scales that range from a single cell to a macroscopic multicellular population, and (ii) create precisely-structured multicellular communities in defined three-dimensional (3D) porous matrices with tunable properties. By combining this approach with mathematical modeling, we have begun to unravel the way in which confinement in a crowded space changes how bacteria spread. Specifically, this study has helped to address the forthcoming three key questions. However, our insights are just the tip of the iceberg, so to speak, and we hope that our results will stimulate further research on the subject.
(1) How Does Confinement Influence Single-cell Motion?
We discovered a new mode of motility (called “hopping and trapping”) that cells exhibit in tight and crowded spaces, in stark contrast to the paradigm of “run-and-tumble” motion that is based on studies in bulk liquid [5] (see Figure 1 and Animation 1). Intriguingly, our results thus far suggest the tantalizing possibility that one can generally understand such out-of-equilibrium systems—as well as other forms of active matter—by using extensions of models that researchers previously developed for “passive,” thermally-equilibrated systems [6, 7]. Further exploring this possibility and creating new mathematical models of single-cell motility in complex spaces is a fruitful direction for future research.
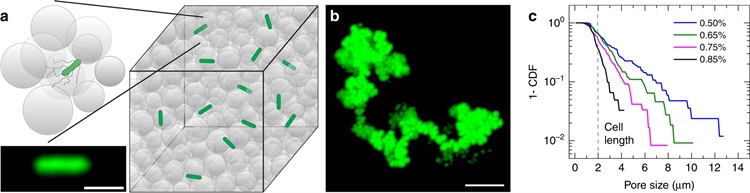
Figure 1. Direct visualization of bacterial motility in three-dimensional (3D) porous media. 1a. Schematic of a 3D porous medium that consists of jammed packings of liquid-infused hydrogel particles, shown as gray circles. Bacteria, shown in green (micrograph shown in the inset), move through the pores between the particles. The scale bar represents 2 micrometers. 1b. Time projection of a 200-nanometer fluorescent tracer particle as it diffuses through the pore space and reveals tortuous channels, each of which is composed of a series of randomly oriented, directed paths. The scale bar represents 5 micrometers. 1c. Complementary cumulative distribution function of the smallest confining pore size for four different porous media with various hydrogel particle packing densities. The percentages indicate the mass fraction of dry hydrogel granules that are used to prepare each medium. The dashed line represents the cell body length of E. coli as a reference. Distributions are exponential, as evidenced by straight lines on log-lin axes. Figure courtesy of [5].
(2) How Does Confinement Influence the Collective Spreading of Multicellular Populations?
Many bacterial populations collectively spread via chemotaxis — biased motion in response to local chemical gradients, which are often established by the cells themselves through the consumption of surrounding nutrients. This process allows the cells to spread as coherent fronts over large distances and colonize new terrain. Our experiments indicate that cells use a fundamentally different mechanism to collectively direct their motion in confinement than they do when in bulk liquid, which suggests that cells employ different mechanisms to bias motion and forage for nutrients in different environments [4]. This realization motivates further studies of chemotaxis in complex settings. We also determined that by promoting cell-solid and cell-cell collisions, confinement markedly alters the dynamics and shape of the migrating population (see Figure 2 and Animations 2 and 3). We developed a simple continuum model that describes these features by coupling bacterial and nutrient dynamics while explicitly incorporating the influence of confinement [2]. Subsequent studies could build upon this work to develop more accurate models of bacterial migration in crowded environments.
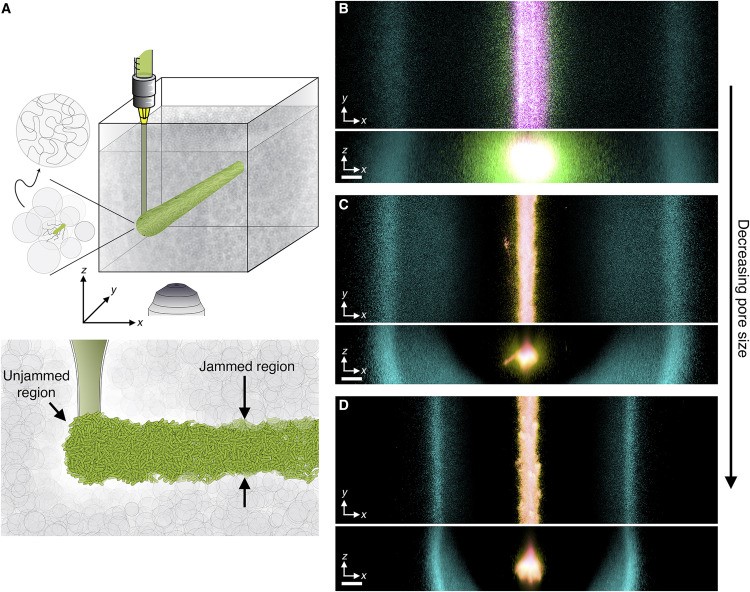
Figure 2. Propagation of chemotactic fronts in three-dimensional (3D) porous media. 2a. Schematic of a cylindrical population of bacterial cells (green cylinder) that is 3D-printed within a porous medium (gray). 2b. Top and bottom panels illustrate bottom-up (\(xy\) plane) and end-on (\(xz\) plane) projections of cellular fluorescence intensity that is measured with 3D confocal image stacks. Images show a section of an initially cylindrical population at three different times (0, 1, and 2.7 h respectively in magenta, yellow, and cyan) as it migrates radially outward in a porous medium. 2c and 2d depict the same experiment in media with progressively smaller pores. Magenta, yellow, and cyan correspond to 0, 1.8, and 10.3 h in 2c and 0, 1.3, and 17.3 h in 2d. All scale bars represent 200 micrometers; a pixel thus corresponds to ~1 cell, indicating that the cells coherently propagate via multicellular fronts over length scales that span thousands of cell body lengths. Figure courtesy of [4].
We also discovered a mechanism that enables bacterial spreading to persist even when a population is confronted with external perturbations, which inevitably arise in complex settings. In particular, we found that chemotaxis allows the bacteria to autonomously smooth even large-scale perturbations — thereby permitting the cells to continue migrating together as a coherent group [3] (see Figure 3 and Animation 4). By integrating experiments with our mathematical model of bacterial spread, we demonstrated that this process arises due to limitations in individual cells’ abilities to sense and respond to the nutrient gradient at different positions along a migrating front [1]. The outcome reveals an unexpected population-scale consequence of individual cells’ transduction of external signals. It will be interesting to extend these results to study the ways in which active and living systems more generally respond to perturbations.
Animation 2. Bottom-up view of chemotactic migration. Animation courtesy of [4].
Animation 3. End-on view of chemotactic migration. Animation courtesy of [4].
(3) How Does Confinement Influence the Assembly and Growth of Non-motile Bacterial Populations?
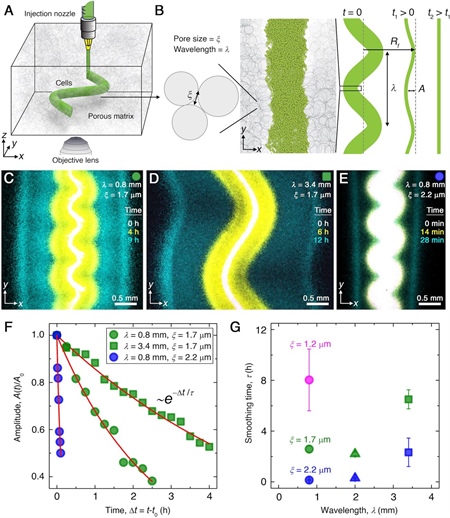
Figure 3. Smoothing of perturbed chemotactic fronts in three-dimensional (3D) porous media. 3a. Schematic of an undulated population (green cylinder) that is 3D-printed within a porous medium (gray). 3b. Two-dimensional \(xy\) slice through the midplane of the population. The starting morphology of the 3D-printed population has undulation wavelength \(\lambda\) and amplitude \(A_0\), as defined by the undulated path that the injection nozzle traces. The cells subsequently swim through the pores between hydrogel particles, with mean pore size \(\xi\). The population thereby migrates outward in a coherent front that eventually smooths. 3c-3e. Bottom-up (\(xy\) plane) projections of cellular fluorescence intensity. Images show sections of three initially undulated populations in three different porous media, each at three distinct times (superimposed white, yellow, cyan), as the cells migrate radially outward. One pixel corresponds to approximately one cell, and the images only reveal a magnified view of the overall population. 3c and 3d demonstrate the influence of varying the undulation wavelength while keeping the mean pore size the same; increasing \(\lambda\) slows smoothing. 3c and 3e demonstrate the influence of varying the pore size while keeping the undulation wavelength the same; increasing \(\xi\) hastens smoothing. 3f. For each experiment in 3c-3e, the normalized undulation amplitude decays exponentially with the time that has elapsed from the initiation of smoothing. 3g. The smoothing time scale that is measured in experiments increases with increasing undulation wavelength and decreasing medium mean pore size, which allows cells to migrate more easily. Figure courtesy of [3].
In many cases, bacteria transition from the motile “planktonic” state to a spatially organized immobilized biofilm; this process is often mediated by the sensing of bacterial-secreted chemical signals known as “autoinducers.” Predicting the competition between motility-based spreading and biofilm formation is notoriously complex. As a first step towards framing this problem in mathematical terms that are experimentally testable, we extended our continuum model of bacterial spreading to incorporate autoinducer dynamics [9]. Doing so established a biophysical threshold that could help predict the onset and extent of biofilm formation across different species and environmental conditions. However, additional research that builds upon this work is necessary to develop more refined models that accurately predict and could thereby guide the control of biofilm formation in diverse settings.
How does an immobilized bacterial population spatially organize its structure as it continues to grow in three dimensions? Our recent efforts demonstrate that bacterial populations adopt a characteristic branched, broccoli-like, self-affine morphology as they grow [8]. This morphology is independent of variations in cell type and environmental conditions — and thus may be characteristic of many populations that grow in natural 3D settings. We elucidated the cause of this morphological instability via a simple continuum model that treats the colony as an “active fluid” whose dynamics are driven by nutrient-dependent cellular growth. In particular, our model describes the manner through which 3D populations inevitably become nutrient-limited in their interiors, thus causing growth to localize at the surface. Spatial variations in surface-level nutrient availability then drive transitions to spatially heterogeneous, rough growth (just as in experiments). But again, this work only scratches the proverbial surface; exploring the dependence of such morphological features on numerous factors—including the mechanical properties of the cells and cell-secreted materials, the structure and mechanical properties of the cells’ surroundings, variations in cell shape and local packing density, and limitations on the space and amount of nutrients that are available to the growing colony—will be an important direction for subsequent research.
Animation 4. Bottom-up view of migration from an undulated cylinder of closely packed E. coli. The cells collectively migrate outward in a front that autonomously smooths the large-scale undulations as it continues to propagate. Animation courtesy of [3].
Future Outlook
Our multiscale visualization and continuum models have helped describe the spread, growth, and spatial organization of bacterial populations in complex 3D environments. Motivated by our findings, we believe that this area of research is rife with opportunities for applied mathematicians to develop sophisticated new models that describe the behavior of bacterial populations and other types of actively moving or growing matter, mediated by interactions with their surroundings. Such developments could motivate novel ways of thinking about transport processes and yield overarching principles to predict and control the organization of bacteria—and active matter in general—in complex, crowded environments. Future models could also potentially facilitate the creation of quantitative guidelines for the control of these dynamics in processes that range from bioremediation and agriculture to drug delivery.
Sujit Datta presented this research during a minisymposium presentation at the 2022 SIAM Conference on the Life Sciences, which took place concurrently with the 2022 SIAM Annual Meeting in Pittsburgh, Pa., this July.
References
[1] Alert, R., Martínez-Calvo, A., & Datta, S.S. (2022). Cellular sensing governs the stability of chemotactic fronts. Phys. Rev. Lett., 128(14), 148101.
[2] Amchin, D.B., Ott, J.A., Bhattacharjee, T., & Datta, S.S. (2022). Influence of confinement on the spreading of bacterial populations. PLOS Comput. Biol., 18(5), e1010063.
[3] Bhattacharjee, T., Amchin, D.B., Alert, R., Ott, J.A., & Datta, S.S. (2022). Chemotactic smoothing of collective migration. eLife, 11, e71226.
[4] Bhattacharjee, T., Amchin, D.B., Ott, J.A., Kratz, F., & Datta, S.S. (2021). Chemotactic migration of bacteria in porous media. Biophys. J., 120(16), 3283-3497.
[5] Bhattacharjee, T., & Datta, S.S. (2019). Bacterial hopping and trapping in porous media. Nat Comm., 10, 2075.
[6] Bhattacharjee, T., & Datta, S.S. (2019). Confinement and activity regulate bacterial motion in porous media. Soft Matter, 48, 9920-9930.
[7] Kurzthaler, C., Mandal, S., Bhattacharjee, T., Löwen, H., Datta, S.S., & Stone, H.A. (2021). A geometric criterion for the optimal spreading of active polymers in porous media. Nat. Comm., 12, 7088.
[8] Martínez-Calvo, A., Bhattacharjee, T., Bay, R.K., Luu, H.N., Hancock, A.M., Wingreen, N.S., & Datta, S.S. (2022). Roughening instability of growing 3D bacterial colonies. Preprint, bioRxiv 2022.05.09.491177.
[9] Moore-Ott, J.A., Chiu, S., Amchin, D.B., Bhattacharjee, T., & Datta, S.S. (2022). A biophysical threshold for biofilm formation. eLife, 11, e76380.
 |
Sujit Datta is an assistant professor and the Director of Graduate Studies of Chemical and Biological Engineering at Princeton University, where his lab examines the dynamics of soft (“squishy”) and living systems in complex environments. He also actively leads outreach efforts in science, technology, engineering, and mathematics to bring together diverse perspectives and provide access to researchers from traditionally underrepresented groups in studies of soft and living systems. |